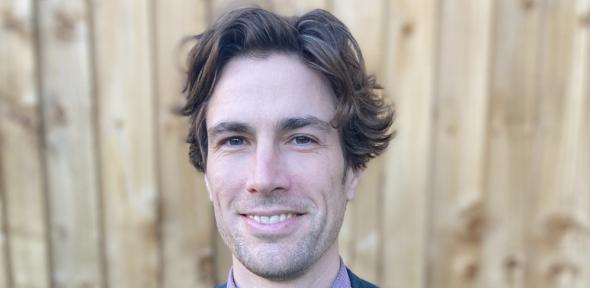
Sean Hartnoll, new Professor of Mathematical Physics at the Department of Applied Mathematics and Theoretical Physics (DAMTP), is connecting extremes. He works on an astonishing link between the theory of black holes and the small-scale physics of materials that can be found back here on Earth.
Black holes are regions of spacetime where the gravitational pull is so strong that not even light can escape. They can form, for example, when a massive star collapses under its own gravity. The first picture of a black hole was taken in 2019 by the Event Horizon Telescope. In it the black hole shows up as a shadow-like dark patch within a surrounding disc of glowing gas.
The theoretical notion of black holes, however, has been around a lot longer. Einstein's theory of gravity — the general theory of relativity — predicts that they should exist and physicists have been aware of this for a long time. Einstein's theory gave physicists the tool to think about black holes even when they cannot observe them.
Black, but not completely
Stephen Hawking, one of DAMTP's most famous members, made huge contributions to the theory of black holes. His mathematics showed that black holes aren't entirely black, but leak a type of radiation that is now named after Hawking. They also have a temperature and something called an entropy — that's a measure of the amount of information in a physical system and is closely linked to temperature. Black holes can therefore be regarded as thermodynamic entities. (You can find out more about Hawking's work on black holes here.)
Science has always been an intellectual pursuit addressing deep questions about the Universe, but it has also always been about practical things. It's only in very lucky times that you get to do both at once. Sean Hartnoll
Black holes share this property with materials we find back here on Earth, such as metals or gases. Since Hawking's initial work on black hole thermodynamics it has become clear that they share other properties too. For example, they have a viscosity and there are analogies to the transport of heat. "If I drop a pebble into a black hole, then from the outside I will see ripples going across the event horizon, and these ripples will spread out in the same way that heat would spread out in a medium, following a diffusion equation," says Hartnoll.
Given that black holes share all these properties with ordinary materials you can then ask, "what kind of a medium is a black hole?"
Strongly quantum
An answer to this question arrived in the late 1990s with the work of the physicist Juan Maldacena. "Through his so-called holographic correspondence Maldacena understood that black holes are a specific type of medium," explains Hartnoll. "You can think of a black hole as a maximally quantum medium."
Unlike general relativity, which describes the world at very large scales, quantum physics describes it at the scales of the tiny particles that make up everything in the world around us. The properties of materials can be understood in terms of these particles. For example metals, such as copper, make good electrical conductors because electrons are able to shoot along the metal like billiard balls rolling down a tube. "The electrons are pretty undisturbed, they just shoot down the wire and conduct electricity for us," says Hartnoll.
This type of behaviour is so straight-forward, you don't actually need quantum mechanics to understand it. The situation is much more tricky in strongly quantum media. "An example would be high temperature superconductors," says Hartnoll. "Here the billions of electrons are all interacting with each other. We can't think of them as balls rolling down a tube." The majority of high temperature superconductors are ceramics. Current applications include MRI scanners, and there are high hopes for their potential in the future.
The interaction between so many electrons means that you need the full power of quantum physics to understand the system — and you soon get stuck. "[These exotic materials] have posed a huge mathematical and computational challenge because we don't have the tools for dealing with situations where a lot of things interact, especially when they interact quantum mechanically."
At the beginning of the 2000s, all this left physicists in a surprising situation. On the one hand, there were very real materials, such as high temperature superconductors, which they didn't have the tools to describe or understand properly. On the other hand, there were black holes, which none of us will (hopefully) ever come into contact with, and which they did have have good mathematical models for. Since both are strongly quantum mediums, it makes sense to use the theory describing one to understand the other.
"It turns out that black holes are models for [strongly quantum media]," says Hartnoll. "You can use black holes as a tool to understand these media." This is a truly amazing connection. "Historically physics is organised by scale. There is astrophysics dealing with big things and particle physic dealing with small things. Here is a case where different scales seem to be related to each other. And that's the starting point for my work."
Connecting extremes
Hartnoll exploits this connection between the scales in both directions. In condensed matter physics he has worked on so-called strange metals. "There are many of these strange metals, but what has been appreciated in the last five years or so is that they share many characteristic properties," he says.
One idea that was inspired from black hole theory involves the time over which processes in strange metals play out. "It turns out that when you take apart the equations that describe these strange metals, what comes out is a characteristic time scale," says Hartnoll. "The conjecture is that this is the shortest [length of] time that makes sense in these many-electron systems — it might not be possible for quantum interactions to happen faster than that." The time scale is called the Planckian time (and it's an analogy to the Planck time in quantum gravity).
"Maybe an organising principle of these unconventional metals is the Planckian time," says Hartnoll. "It's an idea that has motivated many experiments and many new ideas for how to probe strange materials."
In the other direction, Hartnoll has worked on our understanding of the very fabric of space. The kind of matter we are familiar with can exist in different phases — for example gas, liquid or solid — and when it turns from one to the other it does so in a sudden phase transition. "An idea that has grown recently is that space itself is a phase. Maybe the Big Bang was a phase transition, something like a solidification, where space itself came into existence out of something else."
In this budding theory, space is made up of elementary components that are stitched together. The thing that does the stitching is quantum entanglement, a special kind of correlation between quantum particles. "Roughly, the idea is that the quantum state of the system has a lot of components and the more entangled they are the closer they are. This is what builds the very notion of space itself. It's a work in progress, a paradigm, but it's quite exciting and probably true. It's something that I am working on very actively."
Hartnoll was an undergraduate, graduate student and research fellow at DAMTP and is now returning after a 15 year absence — in what he's considering to be exciting times. "The thing about science is that it has always been an intellectual pursuit addressing deep questions about the Universe, but it has also always been about practical things that may eventually improve people's lives. It's only in very lucky times that you get to do both at once. This lack of opposition between the profound and the practical is a very good thing."